The key to silicon anode solutions: Cost
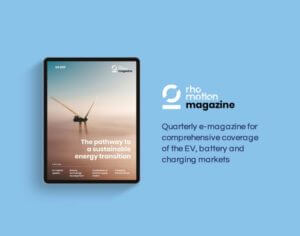
Charging an EV battery is simply storing electrons and lithium ions in the appropriate electrode of the cells in the battery pack. Today, almost all the anode electrodes store electrical energy in natural or synthetic graphite particles coated onto the current collector in large EV cell factories. A few leading EV models (for example Tesla Y, Tesla 3, and Porsche Taycan) contain a small amount (a few percentage points) of silicon oxide additives mixed with the graphite, to increase the cells energy and power density, hence increasing EV range and charging speed for a better driving experience versus graphite-only EVs. Industry experts recognize that consumer demand for even better EVs will drive the amount of silicon to increase progressively to 10-20% in most of the 2025 EV models, and to even higher levels by the end of the decade, accelerating the transition from ICE to EV. But that’s easier said than done. Adding more silicon oxide, for example, is not the answer for both technical and cost reasons. New ways to add more silicon in EV cells is key to the EV competitive race. The economic factors for any silicon solution must be considered across the supply chain: cost of the precursors, capital equipment & manufacturing costs, and impact on the total cell production cost. We will compare three types of silicon solutions to replace current silicon oxide additives:
Type 1: Encapsulating nano-silicon particles into a new type of carbon or polymer matrix mixed with inactive additives and graphite powders (with the goal to replace graphite altogether).
Type 2: New proprietary anode electrodes with no graphite powders
Type 3: Fusing silicon-nanowires directly onto existing commercial graphite without inactive additives and without requiring mixing with graphite powders.
Cost of precursors
Both Type 1 and 2 require a silicon precursor to form silicon particles and a carbon precursor to form a scaffolding and a shell. Metallurgical grade silicon (“MGS”) can be purified and ground into a powder, then coated with amorphous carbon or conductive polymer. Alternatively, MGS is used to produce a pure silane gas (SiH4) which must be converted into nano-silicon particles inside a new carbon matrix because of the very high surface area of these silicon particles. The costs of having to produce a carbon or polymer matrix and having an incomplete conversion of the precursor into silicon anode active material are significant, and the processes produce waste. Type 3 requires only four abundant and inexpensive precursors already scaled-up in mature supply chains: commercial EV grade graphite (EV standard supply chain), silane gas (solar cells standard supply chain), nitrogen (inexpensively extracted from ambient air), and tiny amount (ppm level) of copper catalyst. No new materials are needed to be produced in large quantities and no inactive material is added to the silicon.
Capital equipment and manufacturing costs
The specialized equipment required to produce the additives for Type 1 and 2 is new and custom designed without a reasonable risk-mitigated path to scaleup. When producing small quantities (from one ton to hundreds of tons), the equipment CAPEX amortization costs per kg are high. When contemplating scaling-up to supply meaningful quantities required for millions of EVs new equipment must be designed, built, and tested. This increases risks, upfront investments, and maintenance costs. In addition, the manufacturing high energy consumption costs and the low yields of these solutions come with a significant carbon footprint impact (e.g., waste processing). By contrast, Type 3 manufacturing equipment are standard CVD machines, already part of the very mature solar cell supply chain – designed, built, and sold for years in large quantities by multiple suppliers to the huge solar cell factories. The design costs have already been amortized and the safety, reliability, and the lower maintenance costs are well known. In addition, the use of the copper catalyst to decompose silane into nanosilicon is key to reducing manufacturing costs: more than 99% of the silane is converted into nano-silicon at a lower temperature and the hydrogen exhausted can be re-used. Type 3 relies on a faster, simpler process with no waste and lower electricity costs and smaller carbon footprint. Based on actual measured process metrics, including end-to-end yield losses, the Type 3 variable costs to add silicon nanowires to commercial graphite totals only about $1.6 per kWh added to the energy storage capacity of the graphite. Since EV grade graphite typically cost about $6 per kWh, the cost of the resulting composite anode active materials is lower, and the cost savings increase as the amount of silicon nanowires fused onto the graphite increase.
Total cell production costs
Anode active materials are blended with conductive additives and binders to form a slurry, which is applied onto current collector foils in the large coating machines found in EV cell factories. The anodes are then paired with separators and cathodes and the cell formation cycles are completed before assembly into EV battery packs. The silicon solution choice has a largely underestimated impact on the costs associated with these steps. Type 1 and 2 silicon solutions are responsible for a substantial cost increase of this EV cell manufacturing process. First, slurry mixing requires a departure from the recipes fine-tuned with commercial EV-grade graphite powders for years. This is because these silicon powders introduce multiple new materials and particle sizes, affecting the slurry rheology, the adhesion to the electrode foils, and require new slurry formulations. In addition, these silicon solutions introduce significant new challenges when moving from wet to dry electrode coating. Finally, these silicon solutions lower first cycle efficiency, requiring extra costly cathode materials. Type 2 solutions require new anode machines that are proprietary and not yet produced or used at large scale in EV factories, which increases risks and initial CAPEX costs. By contrast, the Type 3 solution is based on fusing nanosilicon on the same graphite powders already used in preparing slurries and coating electrodes in large EV cell factories. The addition of tiny silicon nanowires onto the micron-sized graphite particles, surprisingly, slightly reduces the BET surface area of the pure graphite substrate. And the size distribution of the particles in the composite powder is identical to the distribution in the pure graphite. Thus, the mixing and slurry rheology are simple to fine-tune and the coating of graphite with fused nano-silicon achieves uniform distribution – whether in large electrode coating machines using wet coating or in the newer, significantly less expensive dry coating processes. The first cycle efficiency of the Type 3 composite is about the same as that of the graphite used as a substrate. This avoids extra cathode costs. Furthermore, in dry electrode processes, the option exists to add a small amount of stabilized lithium powder, which can further increase first cycle efficiency by direct contact pre-lithiation. There is no need for special conductive polymers to ensure the proper electrical pathways for electrons to move in and out of the silicon during cycling. The contact points between the tens of thousands silicon nanowires on each graphite particles are permanent and act like the plug of an extension cord into an outlet (the graphite particle). SEI layers form onto both the silicon nanowires and the graphite particles, but not at the attachment points. Finally, simple formation protocols keep the formation costs to a minimum.
Conclusion
We believe that the 2025 EV models year will determine the winners of the ICE to EV conversion race. Highly disruptive manufacturing processes can’t scale fast enough and are cost prohibitive. Silicon nanowire technology, however, offers the performance, scale, and cost effectiveness to win that race.